From the Laboratory Bench to the Patient’s Bedside: An Update on Clinical Trials With Mesenchymal Stem Cells
ANTONIO GIORDANO, UMBERTO GALDERISI AND IGNAZIO R. MARINO
Mesenchymal Stem Cells (MSCs) are non-hematopoietic multi-potent stem-like cells that are capable of differentiating into both mesenchymal and non-mesenchymal lineages. In fact, in addition to bone, cartilage, fat, and myoblasts, it has been demonstrated that MSCs are capable of differentiating into neurons and astrocytes in vitro and in vivo. MSCs are of interest because they are isolated from a small aspirate of bone marrow and can be easily expanded in vitro. As such, these cells are currently being tested for their potential use in cell and gene therapy for a number of human diseases. Nevertheless, there are still some open questions about origin, multipotentiality, and anatomical localization of MSCs. In this review, we discuss clinical trials based on the use of MSCs in cardiovascular diseases, such as treatment of acute myocardial infarction, endstage ischemic heart disease, or prevention of vascular restenosis through stem cell-mediated injury repair. We analyze data from clinical trials for treatment of osteogenesis imperfecta (OI), which is a genetic disease characterized by production of defective type I collagen. We describe progress for neurological disease treatment with MSC transplants. We discuss data on amyotrophic lateral sclerosis (ALS) and on lysosomal storage diseases (Hurler syndrome and metachromatic leukodystrophy). A section of review is dedicated to ongoing clinical trials, involving MSCs in treatment of steroid refractory Graft Versus Host Disease (GVHD); periodontitis, which is a chronic disease affecting periodontium and causing destruction of attachment apparatus, heart failure, and bone fractures. Finally, we will provide information about biotech companies developing MSC therapy.
J. Cell. Physiol. 211: 27–35, 2007. © 2007 Wiley-Liss, Inc.
What are Mesenchymal Stem Cells?
The microenvironment of mammalian bone marrow is composed of several different elements that support hematopoiesis and bone homeostasis (Muller-Sieburg and Deryugina, 1995; Zhang et al., 2003). It includes a heterogeneous population of cells: macrophages, fibroblasts, adipocytes, osteoprogenitors, endothelial cells (ECs), and reticular cells. Among these, there are also non-hematopoietic stem cells that posses a multilineage potential (Deans and Moseley, 2000; Bianco et al., 2001). These stem cells are commonly indicated as marrow stromal stem cells or mesenchymal stem cells (MSCs). Mesenchymal cells are primordial cells of mesodermal origin giving rise to skeletal muscle cells, blood, vascular and urogenital systems, and to connective tissues throughout the body (Prockop, 1997; Beyer Nardi and da Silva Meirelles, 2006; Sethe et al., 2006). For this reason, the word mesenchymal should be referred to stem cells that are also able to produce blood cells. In practice, however, blood cells derive from a distinct stem cell population present in bone marrow: the hemapoietic stem cells (HSCs) (Prockop, 1997; Beyer Nardi and da Silva Meirelles, 2006; Sethe et al., 2006). MSCs can be hence considered non-hematopoietic multipotent stem-like cells that are capable of differentiating into both mesenchymal and non-mesenchymal lineages. In fact, in addition to bone, cartilage, fat, and myoblasts, it has been demonstrated that MSCs are capable of differentiating into neurons and astrocytes in vitro and in vivo (Pittenger et al., 1999; Bianco and Gehron Robey, 2000; Jori et al., 2005; Beyer Nardi and da Silva Meirelles, 2006) (Fig. 1).
MSCs are of interest because they are easily isolated from a small aspirate of bone marrow and can be expanded through as many as 50 population doublings in about 10 weeks. As such, the cells are currently being tested for their potential use in cell and gene therapy for a number of human diseases. Nevertheless, there are still some open questions about origin, multipotentiality and anatomical localization of MSCs. As far as this latter point is concerned, it has been shown that MSCs can be isolated from different tissues other than bone marrow, which, however, is the primary source for obtaining these stem cells. MSCs have been isolated from adipose tissue, liver, tendons, synovial membrane, amniotic fluid, placenta, umbilical cord, and teeth (Prockop, 1997; Bianco and Gehron Robey, 2000; Beyer Nardi and da Silva Meirelles, 2006; Sethe et al., 2006). Another hot issue is the lack of a single marker to clearly define MSCs. In fact, at present, MSCs are identified through a stem cell populations similar to MSCs but which have been defined with a different nomenclature, such as the bone marrow stromal stem cells, stromal precursor cells, recycling stem cells, marrow isolated adult multineage inducible stem cells (MIAMI cells), and multi-potent adult progenitor cells (MAPC) (Reyes et al., 2001; D’Ippolito et al., 2004; Beyer Nardi and da Silva Meirelles, 2006). MIAMI and MAPC stem cells have a higher proliferative and differentiative potential compared to classical MSCs. It has been suggested that these may represent a more primitive subset of stem cells that could be the common precursor of MSCs and HSCs (Reyes et al., 2001; D’Ippolito et al., 2004; Beyer Nardi and da Silva Meirelles, 2006). If this is the case, then the relationship between these cell populations and the hemoangioblasts that are considered the mesodermal precursors of hematopoietic and EC lineages has to be determined (Park et al., 2005; Sethe et al., 2006) (Fig. 1).
Fig. 1. Diagram of mesenchymal stem cell hierarchy. At top of diagram are indicated the MAPCs and the MIAMI cells that posses a higher proliferativeanddifferentiative potentialcomparedto classicalMSCs.Thesemayrepresent amoreprimitive subset ofstemcells that couldbe the common precursor of MSCs,HSCsand EPCs. In the diagram are indicated themesenchymal and non-mesenchymal cell types that originate from these different classes of stem cells. The dashed lines indicate putative differentiation pathways.
MSCs for Cell Therapy
For more than 100 years, aspirin has served as one of the most effective anti-inflammatory, fever-fighting, pain-relieving drugs on the market. However, its mechanism of action was not discovered until 1971, more than 70 years after aspirin had first appeared on the market. This is not an isolated example, as many times physicians really do not know how their ‘‘tools’’ work. This, however, has not prevented their applications in the clinical setting if there are benefits for patients and there are no or minimal side effects. This type of scenario will probably occur also for cell therapy based on MSCs. These cells have a high expansion potential, genetic stability, can be easily collected and shipped from the laboratory to the bedside and are compatible with different delivery methods and formulations. In addition, MSCs have two other extraordinary characteristics: they are able to migrate to sites of tissue injury and have strong immunosuppressive properties that can be exploited for successful autologous as well as heterologous transplantations (Le Blanc and Pittenger, 2005). In the near future, while scientists will try to learn more about MSC biology, physicians will further develop clinical protocols for MSC-based cell therapy treatments.
MSCs in Hematological Pathologies
Allogenic HSC transplantation could be an effective therapy for several hematological pathologies. However, there could be a number of problems related to treatment, such as infections, bleeding, engraftment failure, and graft versus host disease (GVHD) (Armitage, 1994; Tabbara et al., 2002). GVDH is a form of rejection, where transplanted cells begin to attack host tissues and organs, such as the digestive tract, skin, and liver. It is important to find effective ways to eliminate or at least minimize such serious transplant side effects (Ferrara and Yanik, 2005; Ferrara and Reddy, 2006). MSCs have been shown to have immunosuppressive properties and delay skin graft rejection (Bartholomew et al., 2002; Di Nicola et al., 2002; Le Blanc and Pittenger, 2005). Moreover, MSCs produce cytokines that can support hematopoiesis and potentially enhance marrow recovery following chemotherapy or radiotherapy (Koc and Lazarus, 2001; Le Blanc and Pittenger, 2005). On these bases, several authors have tried to exploit MSCs to facilitate engraftment of HSCs and lessen GVDH. Preliminary studies were carried out by Lazarus et al. (1995). They collected autologous MSCs from patients with hematological cancers in complete remission. MSCs were expanded in culture for 4–7 weeks and then were reinfused intravenously into patients. Patients were grouped in three classes and received 1x106, 5x106, and 10x106/kg MSCs, respectively. No toxicity and adverse reactions were observed, suggesting that MSCs could be useful for transplant treatment (Lazarus et al., 1995). Studies on a patient with severe idiopathic aplastic anemia (SAA) further demonstrated the possible beneficial effect of MSC transplant. A 68-year-old female patient suffered from an end-stage SAA, refractory to conventional therapies. She received an allogenic MSC transplant. Before MSC infusion, the biopsy revealed no hematopoietic tissue, interstitial hemorrhage, edema, adipocytic necrosis, or marrow stromal cells. After transplantation, the majority of these phenomena disappeared, although there was no recovery of hematopoietic tissue, suggesting that allogenic MSC can be safely infused without inducing any side effect and/or GVDH. These studies suggested also that co-infusion of HSCs and MSCs could produce beneficial effects on patients suffering from hematological pathologies (Fouillard et al., 2003). Another interesting result was obtained in a 20-year-old woman suffering from myelogenous leukemia (Lee et al., 2002).
She underwent allogenic HSC and MSC transplantation from her haploidentical father. Peripheral blood mononuclear cells from the father were collected by leukapheresis after pre-treatment with granulocyte colony stimulating factor. The products of leukapheresis were further purified to obtain CD34(þ)HSCs and infused into the patient. Bone marrow aspirate from iliac crest of patient’s father was collected and plated in culture to obtain MSCs. The expanded MSCs were infused after transplantation of HSCs. The patient engrafted rapidly and did not show acute or chronic GVDH. For several months since transplantation, the patient exhibited an enduring trilineage hematological response and complete remission from leukemia. In spite of these positive results, chimeric studies on patients’ bone marrow, carried out several months after transplantation, showed that MSCs were of 100% recipient origin, suggesting that donor MSCs did not engraft. Thus, beneficial effects of allogenic MSC co-infusion could not be clearly attributed to immunosuppressive and/or citokine production (Lee et al., 2002). Lazarus et al. (2005) conducted an exhaustive research on patients suffering from hematological malignancies and treated with co-infusion of HSCs and MSCs. In an open-label, multicenter trial, they enrolled 56 patients that had undergone myeloablative therapy and were responsive to treatment or showed non-progressive disease. MSCs and HSCs were obtained from HLA-identical sibling donors. MSC cultures were prepared starting from 30 ml of donor bone marrow aspirate, while HSCs were obtained either from donor bone marrow aspirate or from peripheral blood stem cells. The planned MSC dose escalation scheme was 1x106, 2.5x106, and 5x106/kg in both patients receiving HSCs from bone marrow or peripheral blood. MSCs were infused 4 h before HSC transplantation. Hematopoietic recovery was prompt for most patients and acute GVDH did not develop in 23 of 46 patients who participated in all phases of the clinical trials. Eleven patients experienced a long relapsed time. These results suggest that introducing culture-expanded MSCs together with HSC transplantation is a safe procedure and could potentially reduce transplant side effects and enhance marrow recovery after myeloablative treatment (Lazarus et al., 2005).
Bone Marrow Stem Cells in Cardiovascular Diseases - Heart diseases
Loss of cardiomyocytes following myocardial infarction induces a contractile dysfunction of heart and the dead cardiac muscle cells are replaced by fibroblasts to form scar tissues. In most circumstances, chronic ischemia persists following infarction and leads to negative remodeling that can cause heart failure and death (Ambrose, 2006). Transplantation of fetal cardiomyocytes or skeletal myoblasts has been proposed as a future method for treatment of heart strokes (Soonpaa et al., 1994; Delcarpio and Claycomb, 1995; Leor et al., 1996; Murry et al., 1996; Taylor et al., 1998; Tomita et al., 1999). Nevertheless, this idea remains unfeasible because of the difficulty in obtaining donor cells and the percentage of failures associated with obtaining sufficient recovery of physiological function in transplanted hearts. Several authors have demonstrated that intracoronary injection of mixed populations of bone marrow stem cells or of MSCs could represent a simple and successful approach to the treatment of heart diseases. An interesting study on this topic was performed by Strauer et al. (2002). They enrolled 20 patients that had suffered from transmural infarction. After right and left catheterization, coronary angiography, and left ventriculography, patients underwent balloon angioplasty and stent implantation. Five to 9 days after acute infarction, bone marrow was aspirated from the ilium of 10 patients and mononuclear cells were isolated with classical Ficoll density separation. Cells were then transplanted into the infarcted zone with the use of a balloon catheter, which was placed within the infarct-related artery. Intracoronary transplantation consisted of six to seven fractional high-pressure infusions, each containing 1.5–4x106 cells. Ex vivo experiments demonstrated that these cells were able to generate mesenchymal cultures. Comparison of the two groups 3 months after cell or standard therapy showed several significant differences. In fact, the infarct region as a percentage of hypokinetic, akinetic, or dyskinetic segments of the circumference of the left ventricle decreased significantly in the cell-transplanted group. Ejection fraction increased in both groups. Perfusion defect was considerably decreased in the cell therapy group as detected by thallium scintigraphy (Strauer et al., 2002). Another study on cell therapy for acute myocardial infarction treatment was carried out by Chen et al. (2004). Sixty-nine patients within 12 h of onset of infarction underwent emergency angiography or angioplasty. Patients were candidates for MSC treatment and were randomized to receive cell transplantation (n¼34) or saline treatment (n¼35) after percutaneous coronary intervention (PCI). Sixty milliliters of bone marrow from patients undergoing cell therapy was aspirated and mononuclear cells were cultured for 10 days to obtain MSCs. At the end of in vitro amplification, cells were collected and used for cell therapy. The infarct-related artery was occluded at the proximal edge of the previous angioplasty and 6 ml of MSC suspension (8–10x109 cells/ml) was injected into the target coronary artery. Control patients received standard saline injections. All patients underwent cardiac echocardiography once a month and positron emission tomography was performed 3 and 6 months after implantation. Electrocardiographic monitoring for 24 h was also recorded 3 months after the procedure. The percentage of hypokinetic, akinetic, and dyskinetic segments decreased significantly in the cell therapy group after 3 months compared to that at the beginning of treatment. This result was obtained to a lesser extent also in the control group. Wall movement velocity over the infarcted area increased significantly in cell therapy-treated patients but not in the control group. Also left ventricular ejection was higher in the cell therapy group compared to controls (Chen et al., 2004).
An interesting randomized trial (called BOOST trial) to assess the effectiveness of intracoronary transfer of autologous bone marrow cells for treatment of acute myocardial infarction was carried out by Wollert et al. (2004). They enrolled 60 patients suffering from acute heart infarction. After PCI, patients were randomly assigned to either a control group that received classical post-infarction treatment or to cell therapy. Bone marrow nucleated cells were collected from patients in the cell therapy group and 4–8 days post PCI were injected (about 24x108) into infarcted artery by a balloon catheter. Changes in left-ventricular end diastolic volumes (LVEDV) index, left-ventricular end systolic volumes (LVESV) index, and left-ventricular mass index did not differ significantly between the control group and bone marrow cell group. However, compared with the control group, patients in the cell therapy group had increased left-ventricular ejection fraction (LVEF) and systolic wall motion 6 months after transplantation. It is noteworthy that there were no differences between the two groups with respect to the number of premature ventricular complexes and the occurrence of non-sustained or sustained ventricular tachycardias by Holter monitoring follow-up at 6 weeks, 3 months, and 6 months. The authors suggested that autologous bone marrow cells can be used to enhance left ventricular functional recovery in patients that had acute heart infarction (Wollert et al., 2004).
A different approach for treatment of myocardial infarction was presented by Katritsis et al. (2005). They acknowledged that intracoronary transplantation of autologous bone marrow-derived mononuclear cells has been shown to improve contractility of infarcted hearts. However, the authors stated that while administration of unpurified mononuclear cells avoids problems associated with cell culture expansion, it inevitably consists of a small percentage of pluripotent cells diluted among a huge amount of committed and differentiated cells. They hypothesized that a bone marrow population consisting of culture-expanded MSCs along with endothelial progenitors (EPCs) also present in marrow stroma would be capable of promoting both myogenesis (by MSCs) and angiogenesis (EPCs) at the infarcted area of the myocardium. The hypothesis relies on several studies suggesting that other cell populations besides hematopoietic stem cells also can give rise to ECs. In fact, adult bone marrow-derived stem/ progenitor cells which are distinct from hematopoietic stem cells, have also been shown to differentiate to the endothelial lineage (Urbich and Dimmeler, 2004). These authors enrolled patients with both recent and old anteroseptal myocardial infarction. All patients had been previously subjected to angioplasty and stent implantation of the left anterior descending artery. In a group of patients (n¼11), the day following PCI, bone marrow aspirates were collected and mononuclear cells were isolated by classical Ficoll separation. Cells were plated in cultures and on day 7, the adherent cells were washed, collected, and transferred to the operating room. The left coronary artery was catheterized for cell transplantation. Two cell suspensions (each containing 1–2x106 cells) were infused distally to the occluding balloon of the catheter. Both in the transplantation group and the controls, there was a trend for improvement in end-diastolic and end-systolic diameter, fraction shortening, ejection fraction, end-diastolic, and end-systolic volume. In 5 out 11 patients in the transplantation group, there was improvement of myocardial contractility in one or more previously non-viable myocardial segments. No one in the control group showed this improvement. Overall evaluation of obtained results indicated that the positive effect of cell therapy on myocardial contractility is mainly seen in patients with recent myocardial infarction (Katritsis et al., 2005). All of the above-described results, along with similar studies (Assmus et al., 2002; Stamm et al., 2003), demonstrate that cell therapy with bone marrow-derived stem cells is feasible, safe, and may contribute to regeneration of myocardial tissue following infarction. Nevertheless, several issues are still controversial: which kind of stem cell is suitable for patients? When should cells be transplanted? How should the viability of transplanted cells be monitored? What is the ideal mechanism of action of stem cells: secretion of growth factors or cell-to-cell interactions?
A study performed by Perin et al. (2003) evaluated the hypothesis that transplants of bone marrow mononuclear cells in patients with end-stage ischemic heart disease may promote neovascularization and may prevent impairment of heart functionality which in turn can lead to myocardial infarction. They enrolled 21 patients, 14 were assigned to the cell therapy group and 7 to the control group. The inclusion criteria for patients were: (i) chronic coronary disease; (ii) left ventricular ejection fraction <40%; (iii) ineligibility for percutaneous or surgical revascularization. For patients undergoing cell therapy treatment, 50 ml of bone marrow was aspirated from the iliac crest and bone marrow mononuclear cells were separated using the Ficoll density procedure. Patients underwent heart catheterization on the left side and electromechanical mapping (EMM) of the left ventricle to target the specific treatment area by identifying viable myocardium. In this area, 15 injections of 2 ml were delivered for a total of ~25x106 cells/patient. All patients underwent a complete non-invasive follow-up (clinical evaluation, ramp tread mill protocol, 2D Doppler echocardiogram, and single photon emission computed tomography analysis) 2 months later and an invasive follow-up (left ventricle angiograms and EMM) after 4 months. Two months after treatment, they observed a significant reduction in total reversible defect and improvement in global left ventricular function within the treatment group and between this and the control group. The 4-month follow-up revealed an improvement in ejection fraction and a reduction in end-systolic volume in the treated patients (Perin et al., 2003). This preliminary study demonstrated that cell therapy treatment could improve myocardial blood flow with associated enhancement of left ventricular functions in patients with severe ischemia and could reduce the risk of heart stroke. The same research team further evaluated the effectiveness of their cell therapy protocol by evaluating patients with severe ischemia 6 and 12 months after transendocardial injection of autologous bone marrow cells. They showed that total reversible defect, detected by SPECT perfusion scanning, was reduced in the cell therapy group compared with controls. Moreover, at 12 months, exercise capacity was significantly improved in cell therapy-treated patients (Perin et al., 2004). These data further support the effectiveness of autologous bone marrow infusion for ischemic cardiomyopathy treatment.
Vascular diseases
Arterial (re)stenosis is a pathophysiological phenomenon that can follow angioplasty, arteriotomy, or by-pass creation in humans and experimental vascular injury in animal models, causing an occlusion of the arterial lumen of variable extension that often requires a new revascularization procedure. Vascular injury, with cell loss in the intima and media tunicae, elastic lamina fragmentation and damage to tissue architecture, leads to excessive pathological repair and remodeling that involves vascular smooth muscle cell (SMC) migration and proliferation, resulting in neointimal hyperplasia (Forte et al., 2001; Xu et al., 2004). Recent evidence has shown that vascular function depends not only on cells within the vessels, but is also significantly modulated by circulating cells derived from the bone marrow. Stem cells hold a great potential for the regeneration of damaged tissues in cardiovascular diseases. In particular, in the past, it was believed that the regeneration of injured endothelium and media in arteries was due to migration and proliferation of neighboring ECs and SMCs. Recent studies clearly indicated that different stem cell populations, derived from bone marrow and characterized by different markers and with different behaviors, contribute to vascular remodeling after injury (Tanaka et al., 2003). On this basis, it has been hypothesized that the restenosis process could be prevented through stem cell-mediated early injury repair. One interesting study was carried out using EPCs. The study is part of the HEALING-FIM (Healthy Endothelial Accelerated Lining Inhibits Neointimal Growth-First In Man) Registry. HEALING I was a single-center, prospective, non-randomized registry trial. It was conducted by Aoki by applying in patients the GenousTM Bio-engineered Rstent (OrbusNeich Company), the first stent designed to accelerate the natural healing response by capturing a patient’s own EPCs from the blood stream (Aoki et al., 2005). Once captured, EPCs rapidly form a protective endothelial layer over the stent, providing protection against thrombus and minimizing restenosis. This stainless steel stent is coated with a murine monoclonal antibody against human CD34.The first published results of this clinical study were obtained on 16 patients and revealed that this coated stent was safe and feasible. On this basis, the group started with the HEALING II study, which included 63 patients at 10 centers in Europe. Whole blood samples were analyzed to quantify the number of EPCs in each patient. Data showed that the EPC titer directly correlated with angiographic outcomes. There were no target lesion revascularizations in patients with normal numbers of circulating EPCs, while patients with low EPCs were affected by restenotic and cardiac events. It should be mentioned that the large majority of patients with normal EPC levels were on statin therapy, while most in the low EPC group were not. Previous studies revealed that statin injection is effective in EPC mobilization (Walter et al., 2002). On the basis of these encouraging results, the HEALING III study has been designed to verify and substantiate these findings and will be conducted in 2006 (Silber, 2006). The HEALING III study will also assess the effect of combining statin therapy and EPC capturing stents.
MSCs for Treatment of Osteogenesis Imperfecta
Osteogenesis imperfecta (OI) is a genetic disease characterized by production of defective type I collagen, the principal protein in bone. OI patients have several painful fractures, retarded bone growth with progressive bone deformation. At present, there is no cure for OI and only one class of drug (bisphosphonates) has been proven to be partially effective (Bembi et al., 1997; Marini and Gerber, 1997). A different approach to treating this disease is the use of cell therapy based on MSCs. In fact, in preclinical experiments carried out on animal models, transplanted MSCs migrated and became incorporated into the bone and muscle of recipient animals (Pereira et al., 1995; Ferrari et al., 1998; Onyia et al., 1998). Therefore, MSC transplants could be useful to correct defects associated with OI. Horwitz et al. (1999) demonstrated the feasibility of allogenic bone marrow transplantation for children with severe OI. Three children with OI were selected for cell therapy. They revealed a mutation of either the COL1A1 or COL1A2 gene, which is associated with severe deforming OI. Patients were intravenously infused with unmanipulated bone marrow cells (5.7–7.5x108 cells/kg) from HLA-identical or single-antigen-mismatched siblings after they received ablative conditioning therapy. Chemoprophylaxis against GVHD consisted of cyclosporine treatment (Horwitz et al., 1999). Engraftment was associated with improvements in bone histology as determined by evaluating specimens of trabecular bones taken before implants and 216 days after transplantation. Before transplantation, the bone sample from one patient contained several disorganized osteocytes, enlarged lacunae, and few osteoblasts. After cell therapy, specimens taken near the site used previously showed a reduced number of osteocytes, linearly organized osteoblasts, and lamellar bone formation. Fluorescence microscopy analysis showed an improved bone formation and mineralization. There was also an increase in the total body mineral content (TBBMC) determined with dual energy X-ray absorptiometry (Horwitz et al., 1999). This preliminary study demonstrated that mesenchymal progenitors in transplanted marrow could migrate to bone in children with OI and give rise to osteoblasts that determined an improvement of bone structure. The clinical significance of this study was, however, questionable. In fact, although the increase in TBBMC along with a decrease in fracture rate observed in some patients, the lack of a long-lasting follow-up and the absence of reliable controls did not make it possible to outline definitive conclusions. In a second study, the authors continued their analysis (Horwitz et al., 2001). Seven children with OI were enrolled for a pilot clinical trial, five of them underwent cell therapy treatment, and two were in the control group. Before treatment, all patients had similar growth rate, typical of children with severe type III OI. The study revealed growth acceleration for the children in the cell therapy group 6 months after the transplantation, in contrast to retarded growth for age-matched controls. With extended follow-up, the growth rate slowed but still exceeded the control rate. The authors suggested that the positive effects of bone marrow transplants were to be ascribed to the integration of competent donor cells of the osteoblastic lineage into the developing bone. However, whether the graft included long-living osteogenic precursors or only committed osteoblasts with a short half-life was unclear (Horwitz et al., 2001). The above-described studies could pave the way to correcting defects associated with OI.
MSCs in Neurological and Inherited Diseases - Amyotrophic lateral sclerosis
MSCs have shown to possess great somatic plasticity since they are capable of differentiating into non-mesenchymal lineages. In fact, it has been demonstrated that MSCs are capable of differentiating into neurons and astrocytes in vitro and in vivo (Pittenger et al., 1999; Bianco and Gehron Robey, 2000; Jori et al., 2005; Beyer Nardi and da Silva Meirelles, 2006). Marrow stem cells have been shown to improve neurological performance in rats with brain ischemia. Moreover, in mice with acid sphingomyelinase deficit, MSC transplants delay the onset of neurological abnormalities and extend their lifespan (Chen et al., 2001; Jin et al., 2002; Zhao et al., 2002). On the basis of these studies Mazzini et al. (2003) initiated a study to verify the efficacy of MSC transplantation in patients with amyotrophic lateral sclerosis (ALS). ALS is a pathology that causes a selective loss of motor neurons leading to a progressive decline in muscle functionality and poor prognosis. Current therapies alleviate only symptoms and there is no cure for this pathology (Mazzini et al., 2003). The research group enrolled seven patients with ALS, showing severe functional impairment of lower limbs and mild impairment of upper limbs. A bone marrow aspirate from each patient was used to prepare MSC cultures that were expanded for 3–4 weeks. Cells were then suspended in autologous cerebrospinal fluid and directly transplanted into the surgically exposed spinal cord at T7–T9 levels. No patients experienced severe adverse events following transplantations. Magnetic Resonance Imaging performed 3 and 6 months following transplantation did not show structural changes of the spinal cord or abnormal cell proliferation when compared with the baseline. Three months after cell implantation, a mild trend toward a slowing down of muscular strength decline was observed in the proximal muscle group of lower limbs in four patients (Mazzini et al., 2003). These preliminary results do not allow us to draw any conclusion about the efficacy of MSC transplants for ALS treatment; nevertheless, they pave the way for further studies and trials aiming to treat neurological diseases.
Hurler syndrome and metachromatic leukodystrophy
There are several forms of mucopolysaccharidosis that are lysosomal storage diseases (Peters et al., 1998; Gieselmann, 2003). Hurler syndrome (MPS 1H), a severe form of mucopolysaccharidosis, is an inherited autosomal recessive disease. In Hurler syndrome, the deficiency in a-L-iduronidase results in accumulation of heparan sulfate and dermatan sulfate into lysosomes. As a consequence, patients show progressive hepatosplenomegaly, cardiac failure, muscle diseases, hydrocephalous and mental retardation. These symptoms lead to death during infancy (Peters et al., 1998). Metachromatic leukodystrophy is an autosomal recessive disease due to the deficiency in arylsulfatase A that produces an accumulation of sulfatides, which in turn causes demyelinization of central and peripheral systems. Demyelinazation induces several severe symptoms, such as tetraplegia, spasticity, mental retardation, and total or partial absence of voluntary activities (Koc et al., 2002; Gieselmann, 2003).
In the early 1990’s, hematopoietic bone marrow transplantations were carried out to ameliorate the life of patients with some lysosomal disorders (Field et al., 1994; Krivit et al., 1999). For example, transplantation of allogenic bone marrows in Hurler syndrome patients was shown to halt progression of liver and heart abnormalities; however, muscle alteration still persisted and even progressed. In addition, marrow transplantation in these patients showed a high incidence of graft failure and morbidity. The efficacy of these transplants is believed to be due to tissue infiltration of donor macrophages that express a normal level of a-L-iduronidase and transfer of enzymes into host cells by endocytosis (Koc et al., 2002). To improve the efficacy of cell transplantation for Hurler syndrome and metachromatic leukodystrophy, Koc et al. infused allogenic MSC in patients suffering from such diseases. MSCs have a multipotential lineage differentiation property. The authors hypothesized that after implantation, MSCs could migrate and differentiate into tissue such as bone, cartilage, peripheral and central nervous system, and repair these tissues (Koc et al., 2002). Six patients with Hurler syndrome and five with metachromatic leukodystrophy, who previously underwent successful bone marrow transplantation from HLA-identical siblings, were enrolled for MSC transplantation. Bone marrow aspirates from original donors were collected and MSC cultures were prepared according to classical protocol. A total of 2–10x106 cells/kg were infused intravenously into patients. The authors did not observe infusion-related toxicity. In four patients with metachromatic leukodystrophy, they observed significant improvement in nerve conduction velocities. However, they did not observe any apparent clinical change in patients, such as improvement of mental and physical conditions. The authors concluded that further evaluations have to be carried out before claiming efficacy or failure of MSC transplants for treatment of mucopolysaccharidosis (Koc et al., 2002).
Can MSC Transplants Improve Recovery of Cancer Patients Undergoing Chemotherapy?
The research team of Prof. Lazarus proposed an interesting application of MSC transplants (Koc et al., 2000). They hypothesized that MSC infusions can improve recovery of cancer patients receiving myeloablative therapy. Breast cancer patients treated with high dose chemotherapy generally have complete and rapid neutrophil and platelet engraftment after peripheral blood progenitor cell (PBPC) transplantation. However, low presence of CD34(þ) stem cells into transplant and bone microenvironment damages increase the risk of delayed engraftment or even its failure. The authors proposed that infusion of autologous MSCs along with PBPC transplantation could improve bone marrow microenvironment and, as a consequence, rate and quality of hematopoietic recovery after myeloablative therapy (Koc et al., 2000). In a Phase I/II clinical trial, they enrolled 32 patients with locally advanced or metastatic breast cancer who were eligible for high-dose chemotherapy and PBPC transplantation. Upon enrollment, 35 days before chemotherapy and PBPC transplants, bone marrow aspirates were collected from patients and MSC cultures were prepared according to classical protocol. MSC cultures contained no detectable breast cancer cells as determined after immunostaining with a cocktail of breast cancer-specific antibodies. Patients received PBPC infusion containing 1.5–39x106 CD34(þ) cells/kg. One or 24 h later, 2.2x106 MSCs/kg were infused intravenously into patients. Hematopoietic engraftment was prompt in all patients with neutrophil and platelet recovery in about 8 days. Bone marrow CFU concentrations recovered to 70% of the baseline by 42 days. All patients were discharged from the hospital and only one patient died within 100 days of the transplant. The authors concluded that MSC infusion at the time of PBPC transplantation is feasible and safe and the prompt hematopoietic recovery suggests thatMSCtreatment may have a positive impact on recovery of patients after high-dose chemotherapy (Koc et al., 2000). To our knowledge, even if these results are of interest, there are no other reports on the use of MSCs for prompt recovery after myeloablative therapy for treatment of solid tumors.
Ongoing Clinical Trials
A look at the website: www.Clinical.Trials.gov of United States National Institute of Health provides information on the current clinical trials based on the use of MSCs. In June 2006, the Christian Medical College of Vellore in India started a single center non-randomized, non-blinded Phase I/II clinical trial (NCT00314483) to study the role of MSCs in the treatment of steroid refractory GVHD. This trial will end in December 2008. Physicians will enroll 25 patients who develop GVDH following an allogenic stem cell transplant. MSCs will be expanded from the donors and will be infused at a dose of 1–2x106 cells/kg. In June 2004, the Translational Research Informatics Center in Japan and other collaborators started a non-randomized, open label, uncontrolled, single group Phase I/II clinical trial (NCT 00221130) to evaluate safety and clinical effects of autologous MSC transplants for periodontitis, which is a chronic disease affecting the periodontium and causing destruction of attachment apparatus of teeth and their loss. This trial is scheduled to end in December of 2008. Ten patients with periodontitis have been enrolled and the study will verify the efficacy of cell transplantation. In detail, an injectable gel, made of a mixture of ex vivo expanded MSCs, osteoblast-like cells, will be delivered in the periodontium of patients. In December 2005, the Rigshospitalet in Denmark started a Phase I/II clinical trial (NCT00260338) to evaluate safety and clinical effects of autologousMSCtransplants in 46 patients with severe chronic myocardial ischemia. This trial will end in November 2009. Patients will be treated with direct intramyocardial injections of ex vivo expanded MSCs. Clinical and objective evaluations will be performed at baseline and at 1-year follow-up. The Hadassah Medical Organization in Israel is scheduled to start a randomized, open label, single group Phase I/II clinical trial (NCT 00250302) for treatment of distal tibial fractures. Scientists will enroll 24 patients with third distal tibia fracture without joint involvement. They will undergo autologous implantation of MSC at the fracture site, which should improve healing by avoiding complications associated with bone grafting.
Companies Developing Mesenchymal Stem Cell Therapy
Biotechnology companies developing stem cell therapy are focused on developing and commercializing human stem cell technology in the emerging field of regenerative medicine to treat degeneration of major organ systems. There are dozens of companies that are trying to develop cell therapy (see for example: www.stem-cell-companies.com, a directory of companies involved in stem cell research and development). However, these companies generally disappoint investors. In part, this is because the stem cell company group comprises a relatively small number of enterprises that are early-stage than the wider biotech sector. As human stem cell research is a relatively new area, companies developing cell therapies face several types of risk and some are not able to manage them, thus becoming highly speculative enterprises. Some risks are well described in a commentary by Giebel (2005). The first type of risk is technology risk. For example, is it possible to differentiate stem cells into fully functional cells, cells that will function exactly like the cells destroyed by the degenerative disease? There is also a manufacturing risk: can companies produce all the cells required under current Good Manufacturing Practices? How much does it cost to produce these cells and how high are the profit margins? Will anybody be able to afford the therapy when you are done? Keeping in mind all these problems, it is easy to predict that only a few companies will survive. Among companies dealing with stem cells, there are some specifically devoted to develop cell therapy based on MSCs. Will they be among the winners of these exciting and important ‘‘biotechnological gamble?’’ Osiris Therapeutics, Inc. is a company that currently has three products in clinical trials, based on MSCs: ProchymalTM, ProvacelTM and ChondrogenTM (www.osiristx.com). Ingredients of these products are adult MSCs from healthy adult volunteer donors and are grown and stored with a proprietary procedure. The objective of ongoing clinical trials with ProchymalTM is to evaluate the safety and efficacy in treating GVHD. GVHD is the greatest complication of allogenic bone marrow transplantation and may affect the digestive system, skin, liver, and other body systems. Very often, it is the major cause of death following transplantation (Ferrara and Yanik, 2005; Ferrara and Reddy, 2006). Clinical trials with ProvacelTM will evaluate its safety and efficacy in treating damaged myocardium following an acute myocardial infarction.
The meniscus is responsible for shock absorption, load transmission, and stability within the knee joint. If this tissue is damaged, surgical removal of the meniscus is the current available therapy (Sweigart and Athanasiou, 2001). ChondrogenTM has shown benefit in animal models of meniscectomy. Clinical trials will be carried out on patients who have undergone standard surgical treatment and will receive an injection of ChondrogenTM into the knee to evaluate safety and effectiveness in ameliorating knee injuries. Mesoblast is an Australian company that is devoted to the production of stem cells to be used in pilot clinical trials in patients with orthopedic and cardiovascular diseases (www.mesoblast.com). The proprietary technology of Mesoblast enables extraction, isolation, and scale-up of mesenchymal type stem cells that they have called mesenchymal precursor cells (MPCs). Their technology is based on the identification of unique markers on the surface of MPCs that enable their extraction and purification from human tissues. Osteoarthritis is an inflammatory disease that results in loss of cartilage at the surface of a joint causing pain and interfering with movement (Arden and Nevitt, 2006; Ge et al., 2006). Acute trauma to healthy joints can have a similar outcome to osteoarthritis. Current therapy for this pathology tries to alleviate painful symptoms but is unable to restore the cartilage lining of joints and thus, there is a progressive degeneration of joint surfaces. Mesoblast’s scientists are carrying out clinical trials based on arthroscopic injection of MPCs to enable regeneration of both cushioning and surface cartilage to relieve pain and restore healthy joints. Scientists at this company are also involved in developing MPC-based cell therapy for bone repair. They have claimed to have technology that can generate both new bone and new blood vessels, enabling greater bone regeneration. They are planning to implant autologous MPCs at the site of bone fractures that will improve healing, eliminating complications associated with bone grafting. In fact, this procedure is greatly limited by a lack of blood supply to the new bone and by the small number of regenerating osteocytes in the graft (Sammarco and Chang, 2002). Researchers at Mesoblast are also developing MPC-based cell therapy for heart failure and peripheral arterial disease. These applications are, however, in a preliminary phase. Another biotech company devoted to developing MSC transplantations is BrainStorm Cell Therapeutics, Inc., which has its headquarters in Israel (www.brainstorm-cell.com). They have developed the product NurOwnTM, the ingredients of which are adult MSCs. NurOwnTMshould be utilized for the treatment of neurodegenerative diseases. In fact, the patent protects a procedure for inducing bone marrow-derived stem cells to differentiate into astrocytes, neurons, and oligodendrocytes. The scientists at BrainStorm Cell Therapeutics, Inc. have transplanted astrocytes, derived from MSCs, into rat models of Parkinson’s disease. Within 2 weeks of cell transplantation, they claim to have observed significant improvement in the characteristic disease behavior, including more than 50% reduction in rotational movements and enhancement in paw reaching capacity. Based on the results of these and other pre-clinical studies, the research team is planning to start clinical trials for treatments of neurological diseases.
Conclusions and Outlook
Over the past years, we have witnessed a growing enthusiasm on the part of scientists and physicians regarding gene therapy and related treatments, but the promise has been overshadowed by many difficulties, especially with regard to the efficacy and safety of delivery of exogenous genes to target cells and tissues by viral vectors. There has been great interest in the antisense oligonucleotide technology that has been applied in a number of clinical trials, even though with inconstant success (Galderisi et al., 1999; Forte et al., 2005). As such, researchers, scientists, physicians, and all professionals in the health care system need to be more cautious when dealing with stem cell therapeutic potentials. However, it seems that some have not learned the lessons arising from previous false promises and errors and still look for the ‘‘magic bullet.’’ Traditional cell therapy is founded on the belief that, when healthy cells are injected into patients, cells will automatically find their way to damaged tissues and stimulate the body’s own healing process. Unfortunately, there are a number of potential side effects of which individuals considering this therapy should be aware. Indeed, cell therapy may be dangerous and some cases of patient deaths directly linked to the therapy have been reported in medical literature. Patients may contract bacterial and viral infections carried by the donor cells, and have experienced life-threatening and even fatal allergic reactions. Donor cells may seriously compromise the immune system. Looking at ongoing clinical trials, it is too early to tell whether all therapies based on stem cells will prove to be clinically effective. Thus, despite extensive research, there are still problems with stem cell therapy, since in many cases, deep and exhaustive studies to find out the exact biology of stem cells are omitted, and there are increasing pressures to start with insufficiently controlled clinical trials. It is very important to address all of these issues.
Acknowledgments
This study was partially supported by NIH grants and Sbarro Health Research Organization grants to A.G.
JOURNAL OF CELLULAR PHYSIOLOGY - 2007 WILEY - LISS, INC .
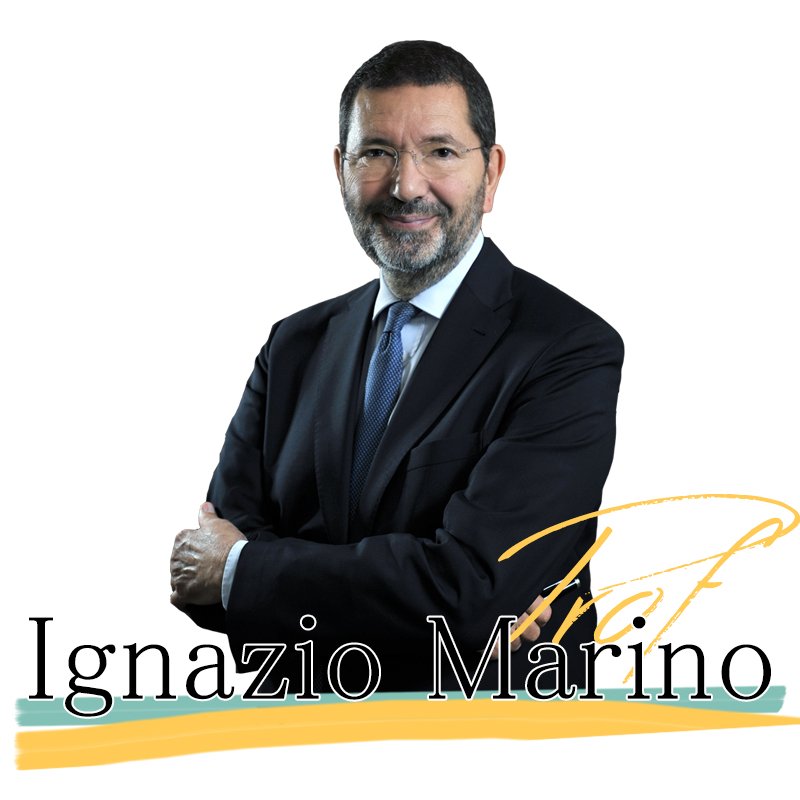